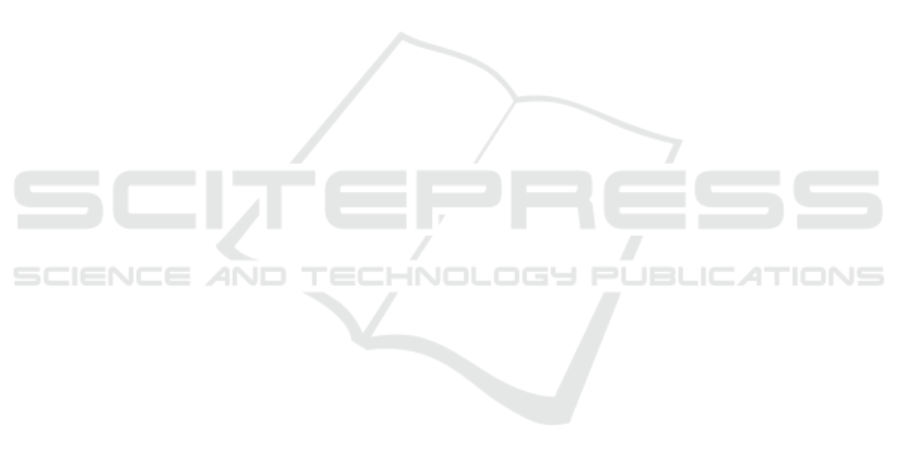
tification and validation by facilitating the identifica-
tion of peptide containing mutations, posttranslational
modifications and/or abnormal fragmentation. Our
study showed that calculating peptides masses using
the whole isotopic collection is more precise than us-
ing only the monoisotopic masses. Hereby, consider-
ing natural isotope would appear to satisfy the criteria
for an optimal quantitative mass spectrometry strat-
egy leading to an accurate peptide/protein identifica-
tion.
REFERENCES
Aebersold, R. and Mann, M. (2003). Mass spectrometry-
based proteomics. Nature, 422(6928):198–207.
Brun, V., Dupuis, A., Adrait, A., Marcellin, M., Thomas,
D., Court, M., Vandenesch, F., and Garin, J. (2007).
Isotope-labeled Protein Standards: Toward Absolute
Quantitative Proteomics. Molecular & Cellular Pro-
teomics, 6(12):2139–2149.
Brun, V., Masselon, C., Garin, J., and Dupuis, A. (2009).
Isotope dilution strategies for absolute quantitative
proteomics. Journal of Proteomics, 72(5):740–749.
Chahrour, O., Cobice, D., and Malone, J. (2015). Stable
isotope labelling methods in mass spectrometry-based
quantitative proteomics. Journal of Pharmaceutical
and Biomedical Analysis, 113:2–20.
Costas-Rodrguez, M., Delanghe, J., and Vanhaecke, F.
(2016). High-precision isotopic analysis of essential
mineral elements in biomedicine: natural isotope ra-
tio variations as potential diagnostic and/or prognos-
tic markers. TrAC Trends in Analytical Chemistry,
76:182–193.
Daron, M., Blamart, D., Peral, M., and Affek, H. (2016).
Absolute isotopic abundance ratios and the accuracy
of 47 measurements. Chemical Geology, 442:83–96.
Deutsch, E. W., Mendoza, L., Shteynberg, D., Farrah, T.,
Lam, H., Tasman, N., Sun, Z., Nilsson, E., Pratt,
B., Prazen, B., Eng, J. K., Martin, D. B., Nesvizh-
skii, A. I., and Aebersold, R. (2010). A guided
tour of the Trans-Proteomic Pipeline. PROTEOMICS,
10(6):1150–1159.
Gerber, S. A., Rush, J., Stemman, O., Kirschner, M. W.,
and Gygi, S. P. (2003). Absolute quantification of
proteins and phosphoproteins from cell lysates by tan-
dem MS. Proceedings of the National Academy of
Sciences, 100(12):6940–6945.
Gray, W. R., Wojcik, L. H., and Futrell, J. H. (1970). Appli-
cation of mass spectrometry to protein chemistry. II.
Chemical ionization studies on acetylated permethy-
lated peptides. Biochemical and Biophysical Research
Communications, 41(5):1111–1119.
Hanke, S., Besir, H., Oesterhelt, D., and Mann, M. (2008).
Absolute SILAC for Accurate Quantitation of Proteins
in Complex Mixtures Down to the Attomole Level.
Journal of Proteome Research, 7(3):1118–1130.
Hunt, D. F., Yates, J. R., Shabanowitz, J., Winston, S.,
and Hauer, C. R. (1986). Protein sequencing by tan-
dem mass spectrometry. Proceedings of the National
Academy of Sciences of the United States of America,
83(17):6233–6237.
Koehler, C. J., Arntzen, M. ., Strozynski, M., Treumann,
A., and Thiede, B. (2011). Isobaric Peptide Termini
Labeling Utilizing Site-Specific N-Terminal Succiny-
lation. Analytical Chemistry, 83(12):4775–4781.
Kristjansdottir, K., Takahashi, S., L., S., and J., S. (2012).
Strategies and Challenges in Measuring Protein Abun-
dance Using Stable Isotope Labeling and Tandem
Mass Spectrometry. In Prasain, J., editor, Tandem
Mass Spectrometry - Applications and Principles. In-
Tech.
Kumar Trivedi, M. (2016). Gas Chromatography-
Mass Spectrometric Analysis of Isotopic
Abundance of <sup>13</sup>C,
<sup>2</sup>H, and
<sup>18</sup>O in Biofield Energy
Treated p-tertiary Butylphenol (PTBP). American
Journal of Chemical Engineering, 4(4):78.
Li, X.-j., Zhang, H., Ranish, J. A., and Aebersold, R.
(2003). Automated Statistical Analysis of Protein
Abundance Ratios from Data Generated by Stable-
Isotope Dilution and Tandem Mass Spectrometry. An-
alytical Chemistry, 75(23):6648–6657.
Listgarten, J. and Emili, A. (2005). Statistical and Com-
putational Methods for Comparative Proteomic Pro-
filing Using Liquid Chromatography-Tandem Mass
Spectrometry. Molecular & Cellular Proteomics,
4(4):419–434.
Mann, M. and Kelleher, N. L. (2008). Precision proteomics:
The case for high resolution and high mass accuracy.
Proceedings of the National Academy of Sciences,
105(47):18132–18138.
Perras, F. A., Chaudhary, U., Slowing, I. I., and Pruski, M.
(2016). Probing Surface Hydrogen Bonding and Dy-
namics by Natural Abundance, Multidimensional,
17
O DNP-NMR Spectroscopy. The Journal of Physical
Chemistry C, 120(21):11535–11544.
Pratt, J. M., Petty, J., Riba-Garcia, I., Robertson, D. H. L.,
Gaskell, S. J., Oliver, S. G., and Beynon, R. J. (2002).
Dynamics of protein turnover, a missing dimension in
proteomics. Molecular & cellular proteomics: MCP,
1(8):579–591.
Schmidt, A., Forne, I., and Imhof, A. (2014). Bioinformatic
analysis of proteomics data. BMC Systems Biology,
8(Suppl 2):S3.
Yates, J. R., Ruse, C. I., and Nakorchevsky, A. (2009).
Proteomics by Mass Spectrometry: Approaches, Ad-
vances, and Applications. Annual Review of Biomedi-
cal Engineering, 11(1):49–79.
The Importance of Considering Natural Isotopes in Improving Protein Identification Accuracy
321