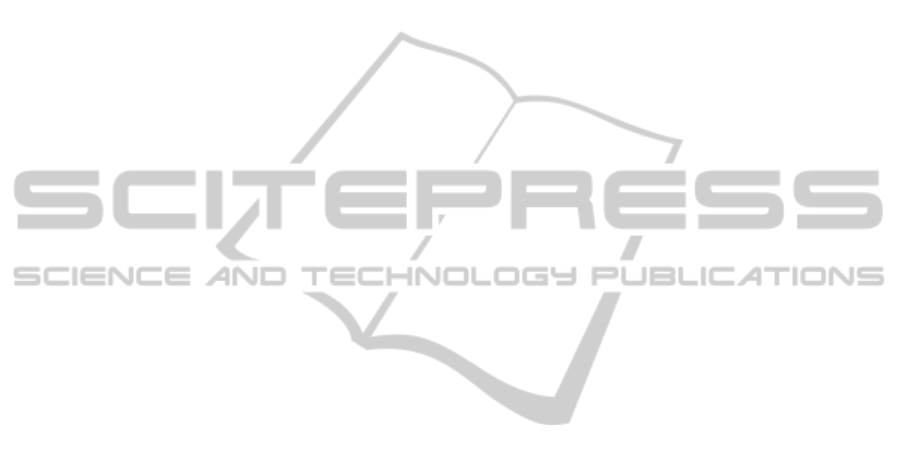
Mechanism for Efficiency Droop” [Phys. Rev. Lett.
110, 177406 (2013)].
Cabalu, J.S., Thomidis, C., Moustakas, T.D., Riyopoulos,
S., Zhou, L., Smith, D.J., 2006. Enhanced internal
quantum efficiency and light extraction efficiency
from textured GaN⁄AlGaN quantum wells grown by
molecular beam epitaxy. J. Appl. Phys. 99, 064904.
doi:10.1063/1.2179120.
Cao, X.A., Arthur, S.D., 2004. High-power and reliable
operation of vertical light-emitting diodes on bulk
GaN. Appl. Phys. Lett. 85, 3971.
doi:10.1063/1.1810631.
David, A., Grundmann, M.J., Kaeding, J.F., Gardner, N.F.,
Mihopoulos, T.G., Krames, M.R., 2008. Carrier
distribution in (0001)InGaN⁄GaN multiple quantum
well light-emitting diodes. Appl. Phys. Lett. 92,
053502. doi:10.1063/1.2839305.
Delaney, K.T., Rinke, P., Van de Walle, C.G., 2009.
Auger recombination rates in nitrides from first
principles. Appl. Phys. Lett. 94, 191109.
doi:10.1063/1.3133359.
Della Sala, F., Di Carlo, A., Lugli, P., Bernardini, F.,
Fiorentini, V., Scholz, R., Jancu, J.-M., 1999. Free-
carrier screening of polarization fields in wurtzite
GaN/InGaN laser structures. Appl. Phys. Lett. 74,
2002. doi:10.1063/1.123727.
Galler, B., Drechsel, P., Monnard, R., Rode, P., Stauss, P.,
Froehlich, S., Bergbauer, W., Binder, M., Sabathil, M.,
Hahn, B., Wagner, J., 2012. Influence of indium
content and temperature on Auger-like recombination
in InGaN quantum wells grown on (111) silicon
substrates. Appl. Phys. Lett. 101, 131111.
doi:10.1063/1.4754688.
Hader, J., Moloney, J.V., Pasenow, B., Koch, S.W.,
Sabathil, M., Linder, N., Lutgen, S., 2008. On the
importance of radiative and Auger losses in GaN-
based quantum wells. Appl. Phys. Lett. 92, 261103.
doi:10.1063/1.2953543.
Hangleiter, A., Hitzel, F., Netzel, C., Fuhrmann, D.,
Rossow, U., Ade, G., Hinze, P., 2005. Suppression of
Nonradiative Recombination by V-Shaped Pits in
GaInN/GaN Quantum Wells Produces a Large
Increase in the Light Emission Efficiency. Phys. Rev.
Lett. 95. doi:10.1103/PhysRevLett.95.127402.
Huang, S., Fan, B., Chen, Z., Zheng, Z., Luo, H., Wu, Z.,
Wang, G., Jiang, H., 2013. Lateral Current Spreading
Effect on the Efficiency Droop in GaN Based Light-
Emitting Diodes. J. Disp. Technol. 9, 266–271.
doi:10.1109/JDT.2012.2225092.
Iveland, J., Martinelli, L., Peretti, J., Speck, J.S.,
Weisbuch, C., 2013. Direct Measurement of Auger
Electrons Emitted from a Semiconductor Light-
Emitting Diode under Electrical Injection:
Identification of the Dominant Mechanism for
Efficiency Droop. Phys. Rev. Lett. 110.
doi:10.1103/PhysRevLett.110.177406.
Kim, H., Cho, J., Lee, J.W., Yoon, S., Kim, H., Sone, C.,
Park, Y., Seong, T.-Y., 2007. Measurements of current
spreading length and design of GaN-based light
emitting diodes. Appl. Phys. Lett. 90, 063510.
doi:10.1063/1.2450670.
Kim, M.-H., Schubert, M.F., Dai, Q., Kim, J.K., Schubert,
E.F., Piprek, J., Park, Y., 2007. Origin of efficiency
droop in GaN-based light-emitting diodes. Appl. Phys.
Lett. 91, 183507. doi:10.1063/1.2800290.
Kioupakis, E., Rinke, P., Delaney, K.T., Van de Walle,
C.G., 2011. Indirect Auger recombination as a cause
of efficiency droop in nitride light-emitting diodes.
Appl. Phys. Lett. 98, 161107. doi:10.1063/1.3570656.
Krames, M.R., Christenson, G., Collins, D., Cook, L.W.,
Craford, M.G., Edwards, A., Fletcher, R.M., Gardner,
N.F., Goetz, W.K., Imler, W.R., Johnson, E., Kern,
R.S., Khare, R., Kish, F.A., Lowery, C., Ludowise,
M.J., Mann, R., Maranowski, M., Maranowski, S.A.,
Martin, P.S., O’Shea, J., Rudaz, S.L., Steigerwald,
D.A., Thompson, J., Wierer, J.J., Yu, J., Basile, D.,
Chang, Y.-L., Hasnain, G., Heuschen, M., Killeen,
K.P., Kocot, C.P., Lester, S., Miller, J.N., Mueller,
G.O., Mueller-Mach, R., Rosner, S.J., Schneider, J.,
Richard P., Takeuchi, T., Tan, T.S., 2000. High-
brightness AlGaInN light-emitting diodes. pp. 2–12.
doi:10.1117/12.382822.
Krames, M.R., Shchekin, O.B., Mueller-Mach, R.,
Mueller, G.O., Zhou, L., Harbers, G., Craford, M.G.,
2007. Status and Future of High-Power Light-Emitting
Diodes for Solid-State Lighting. J. Disp. Technol. 3,
160–175. doi:10.1109/JDT.2007.895339.
Li, C.-K., Wu, Y.-R., 2012. Study on the Current
Spreading Effect and Light Extraction Enhancement of
Vertical GaN/InGaN LEDs. IEEE Trans. Electron
Devices 59, 400–407.
doi:10.1109/TED.2011.2176132.
Morkoç, H., 2008. Handbook of nitride semiconductors
and devices. Wiley-VCH ; John Wiley, distributor],
Weinheim : [Chichester.
Mukai, T., Yamada, M., Nakamura, S., 1999.
Characteristics of InGaN-Based
UV/Blue/Green/Amber/Red Light-Emitting Diodes.
Jpn. J. Appl. Phys. 38, 3976–3981.
doi:10.1143/JJAP.38.3976.
Ni, X., Fan, Q., Shimada, R., Özgür, U., Morkoç, H.,
2008. Reduction of efficiency droop in InGaN light
emitting diodes by coupled quantum wells. Appl.
Phys. Lett. 93, 171113. doi:10.1063/1.3012388.
Piprek, J., Farrell, R., DenBaars, S., Nakamura, S., 2006.
Effects of built-in polarization on InGaN-GaN
vertical-cavity surface-emitting lasers. IEEE Photonics
Technol. Lett. 18, 7–9. doi:10.1109/LPT.2005.860045.
Romanowski, Z., Kempisty, P., Sakowski, K., Stra̧ k, P.,
Krukowski, S., 2010. Density Functional Theory
(DFT) Simulations and Polarization Analysis of the
Electric Field in InN/GaN Multiple Quantum Wells
(MQWs). J. Phys. Chem. C 114, 14410–14416.
doi:10.1021/jp104438y.
Rozhansky, I.V., Zakheim, D.A., 2007. Analysis of
processes limiting quantum efficiency of AlGaInN
LEDs at high pumping. Phys. Status Solidi A 204,
227–230. doi:10.1002/pssa.200673567.
Schubert, M.F., Chhajed, S., Kim, J.K., Schubert, E.F.,
DecorrelationoftheLight-emitting-DiodeInternal-quantum-EfficiencyComponents-StudiesoftheElectron-hole
Concentration-ratioattheActive-regionEdge
45