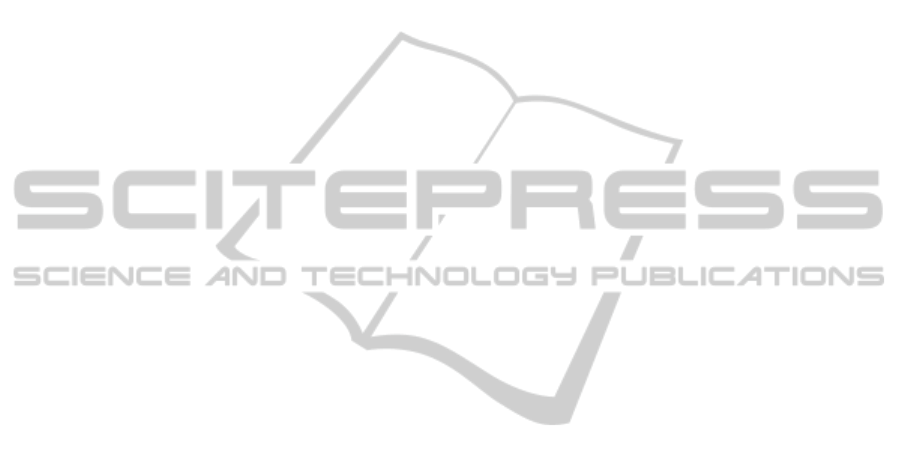
H. Sohlström, K.Gylfason, D. Hill. (2010). Real-time label-
free biosensing with integrated planar waveguide ring-
resonators, Proc. SPIE 7719, 77190B.
Gylfason, K. G., Carlborg, C. F., Kazmierczak, A., Dortu,
F., Sohlström, H., Vivien, L., Barrios, C. A., van der
Wijngaart, W., and Stemme, G., “On-chip temperature
compensation in an integrated slot-waveguide ring
resonator refractive index sensor array”, Opt. Expr.
18(4), 3226–3237 (2010).
C. F. Carlborg, K. B. Gylfason, A. Kamierczak, F. Dortu,
M. J. Bañuls Polo, A. Maquieira Catala, G. M.
Kresbach, H. Sohlström, T. Moh, L. Vivien, J.
Popplewell, G. Ronan, C. A. Barrios, G. Stemme, and
W. van der Wijngaart. (2010). A packaged optical slot-
waveguide ring resonator sensor array for multiplex
label-free assays in labs-on-chips. Lab Chip. 10, p281–
p290.
A. Kazmierczak, F. Dortu, O. Schrevens, D. Giannone, L.
Vivien, D. M. Morini, D. Bouville, E. Cassan, K. g.
Gylfason, H. Sohlström, B. Sanchez, A. Griol, and D.
Hill. (2009) Light coupling and distribution for
Si3N4/SiO2 integrated multichannel single-mode
sensing system. Opt. Eng. 48, (1), 014401.
K. De Vos, I. Bartolozzi, E. Schacht, P. Bienstman, and R.
Baets. (2007). Silicon-on-insulator microring
resonator for sensitive and label-free biosensing. Opt.
Expr. 15, (12), p7610–p7615.
T. Claes, J. G. Molera, K. De Vos, E. Schacht, R. Baets. and
P. Bienstman. (2009) Label-free biosensing with a
slot-waveguide-based ring resonator in silicon on
insulator. J. IEEE Photonics 1, (3), p197–p204.
T. Claes, W. Bogaerts, and P. Bienstman. (2010).
Experimental characterization of a silicon photonic
biosensor consisting of two cascaded ring resonators
based on the Vernier-effect and introduction of a curve
fitting method for an improved detection limit. Optics
Express, 18, (22), p22747.
T. D. Lazzara, I. Mey, C. Steinem, A. Janshoff. (2011).
Benefits and limitations of porous substrates as
biosensors for protein adsorption, Anal. Chem., 83,
(14), p5624-p5630.
M. M. Orosco, C. Pacholski, M.J. Sailor. (2009). Real-time
monitoring of enzyme activity in a mesoporous silicon
double layer. Nature Nanotechnology, 4, p255.
C. K. Tsang, T. L. Kelly, M. J. Sailor, Y. Y. Li, (2012).
Highly Stable Porous Silicon–Carbon Composites as
Label-Free Optical Biosensors. ACS Nano, 6, p10546.
S.D. Alvarez, C. P. Li, C. E. Chiang, I. K. Schuller, M. J.
Sailor. (2009). A Label-Free Porous Alumina
Interferometric Immunosensor. ACS Nano, 3, p3301.
T. Kumeria, M. D. Kurkuri, K. R. Diener, L. Parkinson, D.
Losic, (2012). Label-free reflectometric interference
microchip biosensor based on nanoporous alumina for
detection of circulating tumour cells. Biosensors and
Bioelectronics, 35, (1), 167.
T. Kumeria, D. Losic, (2012). Controlling interferometric
properties of nanoporous anodic aluminium oxide”
Nanoscale Research Letters, 7, (88), p1.
A. A. Yanik, M. Huang, A. Artar, T. Y. Chang, H. Altug.
(2010). Integrated nanoplasmonic-nanofluidic
biosensors with targeted delivery of analytes. Applied
Physics Letters, 96, (2), p021101.
Y. Guo, H. Li, K. Reddy, H.S. Shelar, V.R. Nittoor, X. Fan.
(2011). Optofluidic Fabry-Perot cavity biosensor with
integrated flow-through micro-/nanochannels. Applied
Physics Letters, 98, (4), p041104.
J. Álvarez, P. Bettotti, I. Suárez, N. Kumar, D. Hill, V.
Chirvony, L. Pavesi, J. Martínez-Pastor. (2011).
Birefringent porous silicon membranes for optical
sensing. Opt. Express, 19, (27), p26106.
J. Álvarez, P. Bettotti, N. Kumar, I. Suarez, D. Hill, J
Martínez-Pastor. (2012). Highly-sensitive anisotropic
porous silicon based optical sensors. Proc. SPIE,
8212, (1), p821209.
J. Álvarez, L. Sola, G. Platt, M. Cretich, M. Swann, M.
Chiari, D. Hill, and J. Martínez-Pastor. (2013). Real-
time polarimetric biosensing using macroporous
alumina membranes. Proc. SPIE 8765, Bio-MEMS
and Medical Microdevices, p87650I.
J. Álvarez, L. Sola, M. Cretich, M. J. Swann, K. B.
Gylfason T. Volden, M. Chiari, D. Hill. (2014). Real
time optical immunosensing with flow through porous
alumina membranes. Journal of Sensors and Actuators
B, 202, p834-p839.
G. W. Platt, F. Damin, M. J. Swann, I. Metton, G. Skorski,
M. Cretich, M. Chiari. (2014). Allergen
immobilisation and signal amplification by quantum
dots for use in a biosensor assay of IgE in serum.
Biosensors and Bioelectronics, 52, p82–p88.
M. Cretich, D. Breda, F. Damin, M. Borghi, L Sola, S.M.
Unlu, & M. Chiari. (2010). Allergen microarrays on
high sensitivity silicon slides. Analytical and
bioanalytical chemistry, 398, (4), p1723-p1733.
L. Sola, J. Álvarez, M. Cretich, M. J. Swann, T. Volden, M.
Chiari, D. Hill. (2015). Characterisation of porous
alumina membranes for efficient, real-time, flow
through biosensing. J. Membrane Science, 276, p128–
p135.
J. Álvarez, C. Serrano, D. Hill, and J. Martínez-Pastor.
(2013). Real-time polarimetric optical sensor using
macroporous alumina membranes. Opt. Lett. 38, (7),
p1058-p1060.
M. Iqbal, M. A. Gleeson, B. Spaugh, F. Tybor, W. G. Gunn,
M. Hochberg, T. Baehr-Jones, R. C. Bailey, and L. C.
Gunn. (2010). Label-Free Biosensor Arrays Based on
Silicon Ring Resonators and High-Speed Optical
Scanning Instrumentation. IEEE Journal of Selected
Topics In Quantum Electronics, 16, (3).
K. B. Gylfason, (2010). Integrated Optical Slot-Waveguide
Ring Resonator Sensor Arrays for Lab-on-Chip
Applications. PhD Thesis TRITA-EE 2010:012, KTH-
Royal institute of Technology, Stockholm.
PHOTOPTICS2015-InternationalConferenceonPhotonics,OpticsandLaserTechnology
68