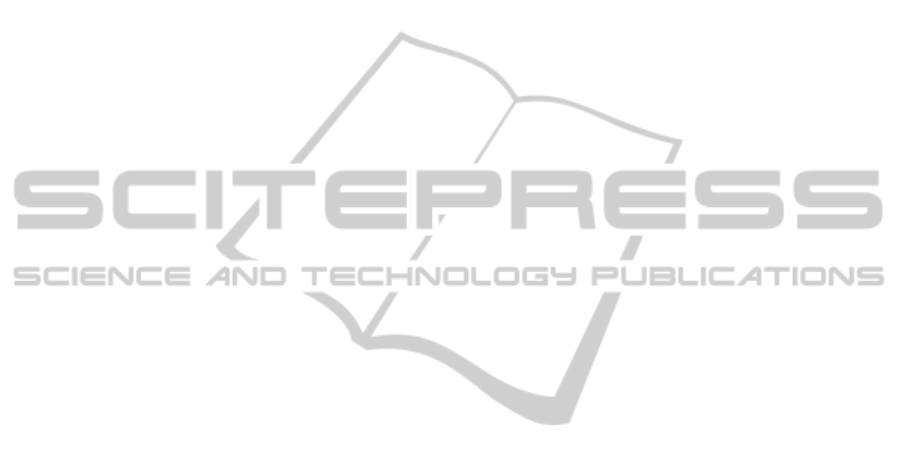
Hadary, A., Olsha, O., Paran, H., Gutman, M.,
Carmon, M., (2008). A prospective, randomized,
controlled, multicenter study of a real-time,
intraoperative probe for positive margin detection in
breast-conserving surgery. Am J Surg 196:483-489
Bard, M. P. L., Amelink, A., Skurichina M., Noordhoek,
Hegt
, V., Duin, R. P., Sterenborg, H. J., Hoogsteden,
H. C., Aerts, J. G., (2006). Optical spectroscopy for
the classification of malignant lesions of the bronchial
tree. Chest 129:995-1001
Chang, S. K., Mirabal, Y. N., Atkinson, E. N., Cox, D.,
Malpica, A., Follen, M., Richards-Kortum, R., (2005).
Combined reflectance and fluorescence spectroscopy
for in vivo detection of cervical pre-cancer. J Biomed
Opt 10:024031
Georgakoudi, I., Jacobson, B. C., Van Dam, J., Backman,
V., Wallace, M. B., Muller, M. G., Zhang, Q.,
Badizadegan, K., Sun, D., Thomas, G. A., Perelman,
L. T., Feld, M. S., (2001). Fluorescence, reflectance,
and light scattering spectroscopy for evaluating
dysplasia in patients with Barrett's esophagus.
Gastroenterology 120:1620-1629
Haka, A. S., Volynskaya, Z., Gardecki, J. A., Nazemi, J.,
Lyons, J., Hicks, D., Fitzmaurice, M., Dasari, R. R.,
Crowe, J. P., Feld, M. S., (2006). In vivo margin
assessment during partial mastectomy breast surgery
using raman spectroscopy. Cancer Res 66:3317-3322
Hamberg, L. M., Hunter, G. J., Kierstead, D., Lo, E. H.,
González, R. G., Wolf, G. L., (1996). Measurement of
Cerebral Blood Volume with Subtraction Three-
dimensional Functional CT. AJNR Am J Neuroradiol
17:1861-1869
Keller, M. D., Majumder, S. K., Kelley, M. C., Meszoely,
I. M., Boulos, F. I., Olivares, G. M., Mahadevan-
Jansen, A., (2010). Autofluorescence and Diffuse
Reflectance Spectroscopy and Spectral Imaging for
Breast Surgical Margin Analysis. Lasers Surg Med
42:15-23
Krishnaswamy, V., Hoopes. P. J., Samkoe, K. S., O’Hara,
J. A., Hasan, T., Pogue, B. W., (2009). Quantitative
imaging of scattering changes associated with
epithelial proliferation, necrosis, and fibrosis in tumors
using microsampling reflectance spectroscopy. J
Biomed Opt 14:014004
Mino-Kenudson, M., Brugge, W. R., Puricelli, W. P.,
Nakatsuka, L. N., Nishioka, N. S., Zukerberg, L. R.,
Misdraji, J., Lauwers, G. Y., (2005). Management of
superficial Barrett's epithelium-related neoplasms by
endoscopic mucosal resection: clinicopathologic
analysis of 27 cases. Am J Surg Pathol 29:680-686
Muller, M. G., Georgakoudi, I., Zhang, Q. G., Wu, J.,
Feld, M. S., (2001). Intrinsic fluorescence
spectroscopy in turbid media: disentangling effects of
scattering and absorption. Appl Opt 40:4633-4646
Muller, M. G., Valdez, T. A., Georgakoudi, I., Backman,
V., Fuentes, C., Kabani, S., Laver, N., Wang, Z.,
Boone, C. W., Dasari, R. R., Shapshay, S. M., Feld,
M. S., (2003). Spectroscopic detection and evaluation
of morphologic and biochemical changes in early
human oral carcinoma. Cancer 97:1681-1692
Novis, D. A., Zarbo, R. J., (1997). Interinstitutional
comparison of frozen section turnaround time. A
College of American Pathologists Q-Probes study of
32868 frozen sections in 700 hospitals. Arch Pathol
Lab Med 121:559-67
Ramanujam, N., Brown, J. Q., Bydlon, T. M., Kennedy, S.
A., Richards, L. M., Junker, M. K., Gallagher, J.,
Barry, W. T., Wilke, L. G., Geradts, J. (2009).
Quantitative Spectral Reflectance Imaging Device for
Intraoperative Breast Tumor Margin Assessment. In:
31st Annual International Conference of the IEEE
Engineering in Medicine and Biology Society.
EMBS’09, Minneapolis, Minnesota, USA, Sept. 2-6,
2009, pp 6554-6556
Tunnell, J. W., Desjardins, A. E., Galindo, L.,
Georgakoudi, I., McGee, S. A., Mirkovic, J., Mueller,
M. G., Nazemi J., Nguyen, F. T., Wax, A., Zhang, Q.
G., Dasari, R. R., Feld, M. S., (2003). Instrumentation
for multimodal spectroscopic diagnosis of epithelial
dysplasia. Technol Cancer Res Treat 2:505-514
Volynskaya, Z., Haka, A. S., Bechtel, K. L., Fitzmaurice,
M., Shenk, R., Wang, R., Nazemi, J., Dasari, R. R.,
Feld, M. S., (2008). Diagnosing breast cancer using
diffuse reflectance spectroscopy and intrinsic
fluorescence spectroscopy. J Biomed Opt 13:024012
Yu, C., Lau, C., O’Donoghue, G., Mirkovic, J., McGee,
S., Galindo, L., Elackattu, A., Stier, E., Grillone, G.,
Badizadegan, K., Dasari, R. R., Feld, M. S., (2008).
Quantitative spectroscopic imaging for noninvasive
early cancer detection. Opt Express 16:16227-16239
Zonios, G., Perelman, L. T., Backman, V. M., Manoharan
R., Fitzmaurice, M., Van Dam, J., Feld, M. S., (1999).
Diffuse reflectance spectroscopy of human
adenomatous colon polyps in vivo. Appl Opt 38:6628-
6637
DEVELOPMENT OF AN EX VIVO QUANTITATIVE SPECTROSCOPIC SCANNER
279