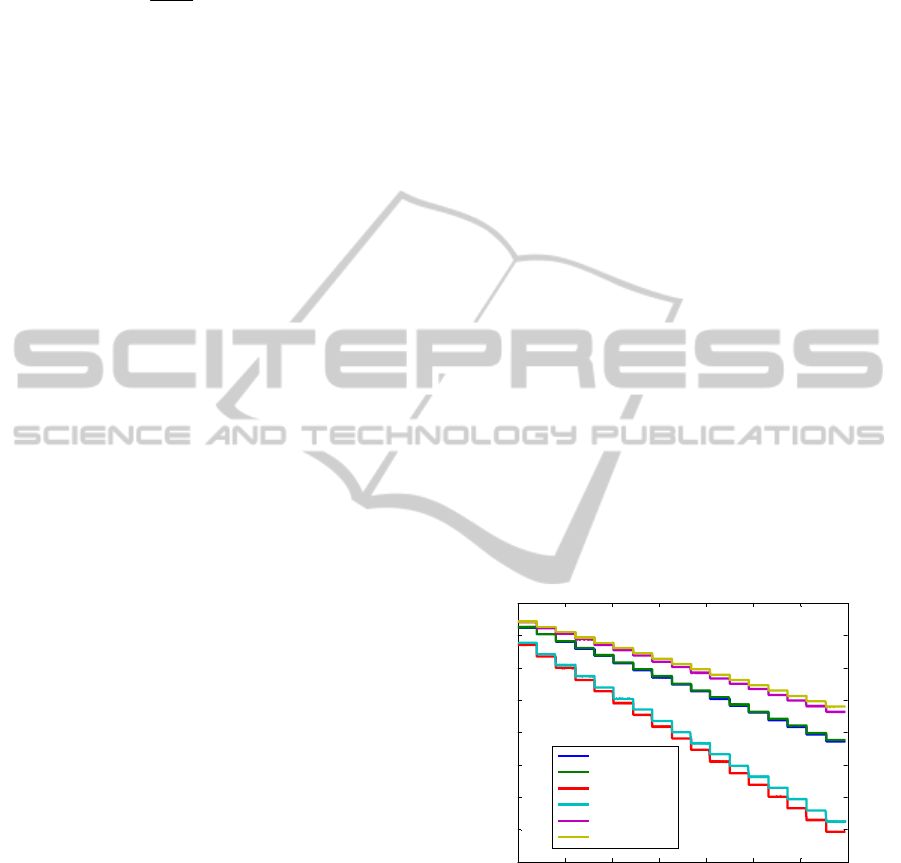
normalized with the factor 1/DC
2
. The normalized
perfusion formula can then be expressed as:
()
noise
PerfPerf
DC
M
Perfusion −=
2
(4)
2.4 In vitro Validation
In vitro validation was performed in order to
evaluate the performance of the prototype to
different scatterer concentrations and velocities.
For in vitro validation two phantoms have been
used. One possesses six layers of Teflon
®
microtubes with internal diameter of 0.30 mm and
an external diameter of 0.76 mm. The other phantom
consists of two glued acrylic plates with a 4×5 mm
2
excavated depression in one of them, used to study
the linearity of the prototype related to different
scatterer velocities and concentrations. Milk was
pumped through the microtube, in the Teflon
®
phantom, or through the depression in the acrylic
phantom. Commercial skimmed milk has been
chosen as a moving fluid, because it has various
components that act as scatterers (Waterworth et al.,
1995).
The milk was pumped with a motorized syringe
at 1.56, 3.12, 4.68, 6.25, 7.78 and 9.35 mm/s in the
Teflon® phantom and at 0, 0.5, 1.0, 1.5, 2.0, 2.5,
3.0, 3.5, 4.0, 4.5, 5.0, 5.5 and 6.0 mm/s in the acrylic
phantom. Measurements were taken with milk, and
with two different aqueous milk solutions (50% and
25%) during ten minutes with Perimed probe
positioned perpendicular to the surface of the
phantom. The mean signal for each velocity and
concentration was computed for three minutes of
blood perfusion signal and a linear regression study
was performed.
The same protocol was executed with the
commercial Periflux 5000 flowmeter, from Perimed,
and with the prototype in order to compare the two
flowmeters.
2.5 In vivo Validation
The prototype was also tested in vivo in healthy non-
smoking subjects. The subjects were asked to refrain
from drinking coffee during the measurements day.
Perfusion has been recorded during thirty three
minutes in the forearm with the subjects in the
supine position: baseline blood flux was recorded for
20 min. Then, an arterial occlusion test was
performed with a pressure cuff placed around the
upper limb, inflated for 3 min at 200 mmHg. The
cuff was then released to obtain a post-occlusive
hyperemia and the signal was recorded during
10 min after the release of the occlusion.
For each subject, the protocol was repeated using
the three laser diodes existing in the prototype: 635,
785 and 830 nm, and also for the commercial
flowmeter, Periflux 5000 from Perimed, with the
probe positioned in the same position during the four
measurements.
The Ethics Committees of the Centro Cirúrgico
de Coimbra (CCC) in Portugal, approved this study.
Informed consent was obtained from the subjects
before the recordings were made. The measurements
were made in 20 subjects and the preliminary results
are presented.
3 RESULTS AND DISCUSSION
3.1 Calibration
The steps obtained with our prototype during the
calibration with the 830 nm LED are shown in figure
2 where a pair of signals was obtained for each
photodetector (as the detection system is made by
bi-cell photodetetors). It can be seen that the
intensity level pairs diverge when the voltage
increases. This is due to the lack of alignment
between the photodetectors and the LEDs.
0 0.5 1 1.5 2 2.5 3 3.5
x 10
5
-8
-7
-6
-5
-4
-3
-2
-1
0
number of points
Voltage steps (V)
bi-cell PD 1 A1
bi-cell PD 1 A2
bi-cell PD 2 A1
bi-cell PD 2 A2
bi-cell PD 3 A1
bi-cell PD 3 A2
Figure 2: Photocurrent generated by the three bi-cell
photodetectors during calibration: PD 1 A1 and A2 are the
PD 1 signals, PD 2 A1 and A2 are the PD 2 signals, PD 3
A1 and A2 are the PD 3 signals.
The detector noise perfusion curve is presented
in figure 3. The slope of the curves are (-6.86, -6.67
and -6.73)x10
-5
for PD 1, PD 2 and PD 3,
respectively and the y-axes value are (0.157, 0.165
and 0.162)x10
-3
with a coefficent of determination,
R
2
, close to one.
BIODEVICES 2012 - International Conference on Biomedical Electronics and Devices
156