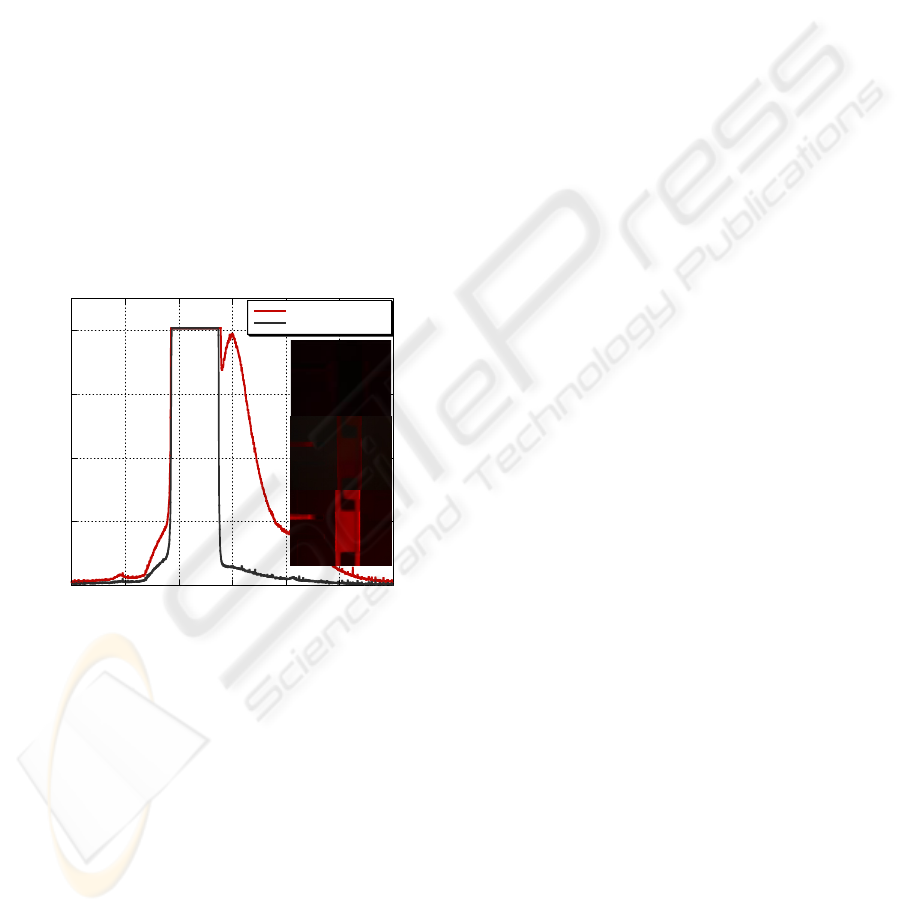
For the DNA hybridization experiments, the
chitosan surface functionalized with probe DNA was
exposed to matching or mismatching DNA solutions
for 30 min at room temperature. In order to
emphasize the selectivity, the mismatching DNA
was twice as concentrated (8 μM) as the matching
DNA (4 μM). Both DNA sequences were labeled at
the 5’ end with AlexaFluor 633 fluorophore. The
response was analyzed with a fluorescence
microscope and a spectrum analyzer.
Fig. 6 displays the intensity response to both
matching and mismatching DNA sequences. A
strong emission signal with a peak at about 650 nm
is demonstrated only for the matching DNA
sequence. The images on the right of the figure
display the fluorescence microscope images of the
sensor area after subjection to both the matching and
mismatching DNA. These results were also
demonstrated to be repeatable using the same device
by washing away the target DNA with a 4 M urea
solution at 95
o
C for 30 min and reintroducing the
DNA samples.
0
1000
2000
3000
4000
500 550 600 650 700 750 800
1-Matching DNA
1-Mis-Matching DNA
INTENSITY [a.u.]
WAVELENGTH [nm]
(a)
(b)
(c)
Figure 6: Collected output spectra demonstrating
successful DNA hybridization. Inset: fluorescence
microscope images of device: (a) after probe DNA
attachment (b) after mismatching target DNA (c) after
exposure to matching target DNA.
5 CONCLUSIONS
Chitosan enables a wide range of applications due to
its unique structure and relative abundance in nature.
We have reported the successful design, fabrication
and testing of two distinct MEMS biosensors which
utilize chitosan as a functionalization layer. Our
microcantilever has a deposited film of chitosan on
its surface to facilitate attachment of probe DNA. Its
structure allows for highly sensitive detection due to
mass loading. Our biophotonics sensor utilizes a
novel sidewall pattern of chitosan to improve
fluorescence emission capture into a planar
waveguide. DNA hybridization experiments have
been successfully performed with both devices. By
bridging the world of MEMS with the world of
biology through mechanical or optical detection,
chitosan forms the missing link allowing for more
robust and selective biological sensors.
ACKNOWLEDGEMENTS
This work was funded by the Laboratory for
Physical Sciences, the National Science Foundation,
and the R. W. Deutsch Foundation.
REFERENCES
Badilita, V., Shamim, I., Yi, H., Koev, S. T.,
Gerasopoulos, K. & Ghodssi, R. (2007) Chitosan-
Mediated Biomems Platform For Optical Sensing. The
14th International Conference On Solid-State Sensors,
Actuators, And Microsystems (Transducers '07). Lyon,
France.
Geng, R., Zhao, G., Liu, M. & Li, M. (2008) A Sandwich
Structured Sio2/Cytochrome C/Sio2 On A Boron-
Doped Diamond Film Electrode As An
Electrochemical Nitrite Biosensor. Biomaterials, 29,
2794.
Koev, S. T., Powers, M. A., Yi, H., Wu, L.-Q., Bentley,
W. E., Rubloff, G. W., Payne, G. F. & Ghodssi, R.
(2007) Mechano-Transduction Of Dna Hybridization
And Dopamine Oxidation Through Electrodeposited
Chitosan Network. Lab On A Chip, 7, 103-111.
Mizutani, F. (2007) Biosensors Utilizing Monolayers On
Electrode Surfaces. Sensors And Actuators B, 130, 14-
20.
Powers, M. A., Koev, S. T., Schleunitz, A., Yi, H.,
Hodzic, V., Bentley, W. E., Payne, G. F., Rubloff, G.
W. & Ghodssi, R. (2005) A Fabrication Platform For
Electrically Mediated Optically Active
Biofunctionalized Sites In Biomems. Lab On A Chip,
5, 583-586.
Yi, H., Wu, L.-Q., Bentley, W. E., Ghodssi, R., Rubloff,
G. W., Culver, J. N. & Payne, G. F. (2005)
Biofabrication With Chitosan. Biomacromolecules, 6,
2881-2894.
BIODEVICES 2009 - International Conference on Biomedical Electronics and Devices
112