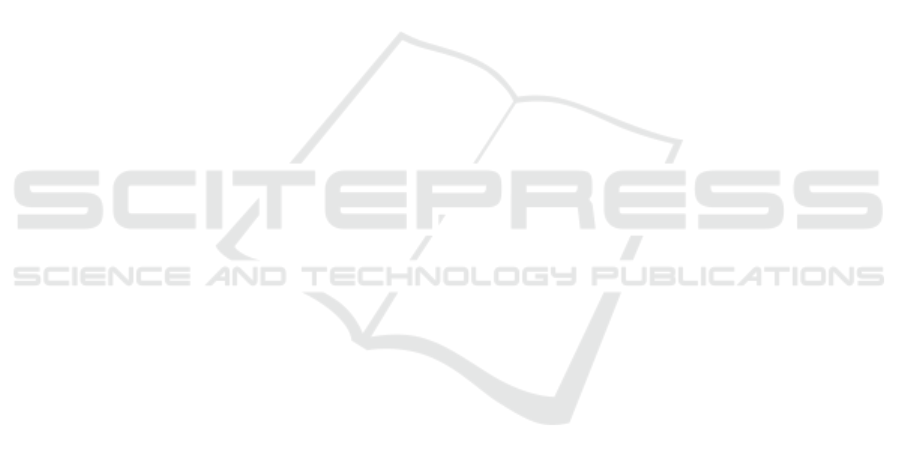
Numerical Study on the Gas-liquid Transportation in
μDMFC Anode Flow Channel with Different Wettabilities
Design
M M Li
*
, Z Li, J Geng and J H Shi
Nanjing University of Aeronautics and Astronautics, Nanjing, 210016, China
Corresponding author and e-mail: M M Li, limiaomiao@nuaa.edu.cn
Abstract. The gas-liquid flow in anode flow channel of micro direct methanol fuel cell
(μDM FC) is simu lated using the VOF method. The effect of channel sidewalls wettability on
the gas-liquid transportation is investigated. Results indicate that improving hydrophilicity of
the sidewall could promote removal of CO
2
bubbles when the wettability of the sidewall
keeps the same everywhere. When the wettability of the sidewall changes with height, the
hydrophilic/neutral combinations have the highest pressure drop, but the
hydrophilic/hydrophobic combinations have the lowest gas fraction in the channel.
Co mparing the two aspects, a conclusion was drawn that sidewalls with hydrophobic upside
and hydrophilic downside facilitate the CO
2
bubble removal and liquid transporting to GDL.
1. Introduction
In recent years, micro direct methanol fuel cell (μDMFC) based on MEMS technology is considered
as a promising power source candidate for portable electronic equipments due to its advantages such
as low temperature operation, high energy conversion, simple structure and convenience of refilling
the liquid fuel [1-3].
The electrochemical reaction taking place in the μDMFC anode is described as follows:
6e6HCOOHOHCH
2
RuPt
23
(1)
As shown in Figure 1, when the μDMFC is in operation, carbon dioxide (CO
2
) bubbles are
generated on the surface of the anode catalyst layer because of the oxidation of the carbon in the
methanol. The reaction-produced CO
2
bubbles emerge from the micro-pores of the gas diffusion
layer (GDL) and transport into the sub-micrometer anode channel, and then move along the flow
channel and out of the fuel cell, which lead to a liquid-gas two-phase flow in the anode flow field of
μDMFC. The CO
2
bubbles could block the channel if not removed efficiently. In that case, not only
less fuel reaches the catalyst layer but also the reaction sites are occupied by the bubbles, resulting in
a decline of the performance of the μDMFC. Hence the effective removal of CO
2
in the anode micro
channels plays - a critical role in the performance of the μDMFC.
Recently, researchers focus their attention on optimizing the channel by changing the wettability
of the surfaces. Zhang et al.
[4] investigated the effect of the wettabilities of the anode GDLs on CO
2
removal on these anode GDLs, and the visualizations of CO
2
gas bubbles dynamics on the anodes
shows that uniform CO
2
gas bubbles with smaller size formed on hydrophilic anode GDLs, and
Li, M., Li, Z., Geng, J. and Shi, J.
Numerical Study on the Gas-liquid Transportation in µDMFC Anode Flow Channel with Different Wettabilities Design.
In Proceedings of the International Workshop on Materials, Chemistry and Engineering (IWMCE 2018), pages 207-214
ISBN: 978-989-758-346-9
Copyright © 2018 by SCITEPRESS – Science and Technology Publications, Lda. All rights reserved
207