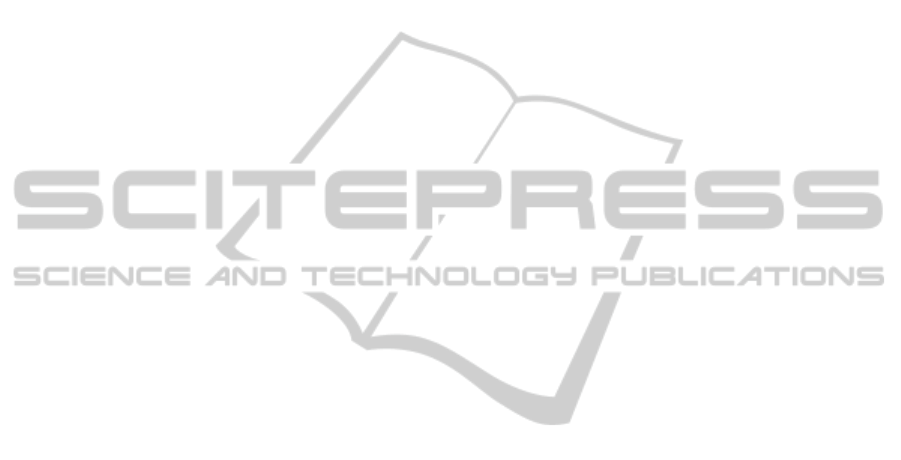
fabricated by reactive ion etching (RIE) techniques
in a standard CMOS process line. Electric field
distributions were calculated. Temperature changes
within the device were determined by optical
microscopy to about 5 °C, which is well compatible
with biomedical applications.
Nanospheres with diameters of 20 nm and
200 nm suspended in water were immobilized at the
electrode tips. For particles larger than the
electrodes, immobilization of single objects was
demonstrated. The procedure was performed within
a few tens of seconds. Any chemical modifications
of suspended particles, dissolved molecules or
surfaces could be avoided.
The demonstrated use of silicon based
nanoelectrode arrays for the dielectrophoretic
immobilization of particles and molecules opens a
novel way for nanoparticle separation and for the
preparation of miniaturized biosensors.
ACKNOWLEDGEMENTS
This work was supported by the grant from the
federal state of Brandenburg and the European
Regional Development Fund.
REFERENCES
Widdershoven, R., Van Steenwinckel, D., Überfeld, J.,
Merelle, T., Suy H., Jedema, F., Hoofman, R., Tak, C.,
A. Sedzin, B. Cobelens, E. Sterckx, R. van der Werf,
K. Verheyden, M. Kengen, F. Swartjes, F. Frederix
IEDM Proceedings 2010.
Martinez-Duarte, R., Electrophoresis 2012, 33, 3110.
Yamamoto, T.; Fujii, T.; Nanotechnology 2007, 18,
495503.
Pethig, R., Biomicrofluidics 2010, 4, 022811.
Diao, J. J., Cao Q., AIP Advances 2011, 1, 012115.
Nakano, A., Ros, A., Electrophoresis 2013, 34, 1085.
Hölzel, R., Calander, N., Chiragwandi, Z., Willander, M.,
Bier, F., Phys. Rev. Lett. 2005, 128102.
Mehr, W., Wolff, A., Frankenfeld, H., Skaloud, T.,
Höppner, W., Bugiel, E., Lärz, J., Hunger, B., Microel.
Engineering 1996, 30, 395-398.
Erickson, H. P, Biological Proc. Online 2009, 11, 32.
Squire, P.G., Moser, P., O’Konski, C.T., Biochemistry,
1968, 7, 4261.
Seger-Sauli, U., Panayiotou, M., Schnydrig, S., Jordan,
M., Renaud, P., Electrophoresis 2005, 26, 2239.
BIODEVICES2014-InternationalConferenceonBiomedicalElectronicsandDevices
180