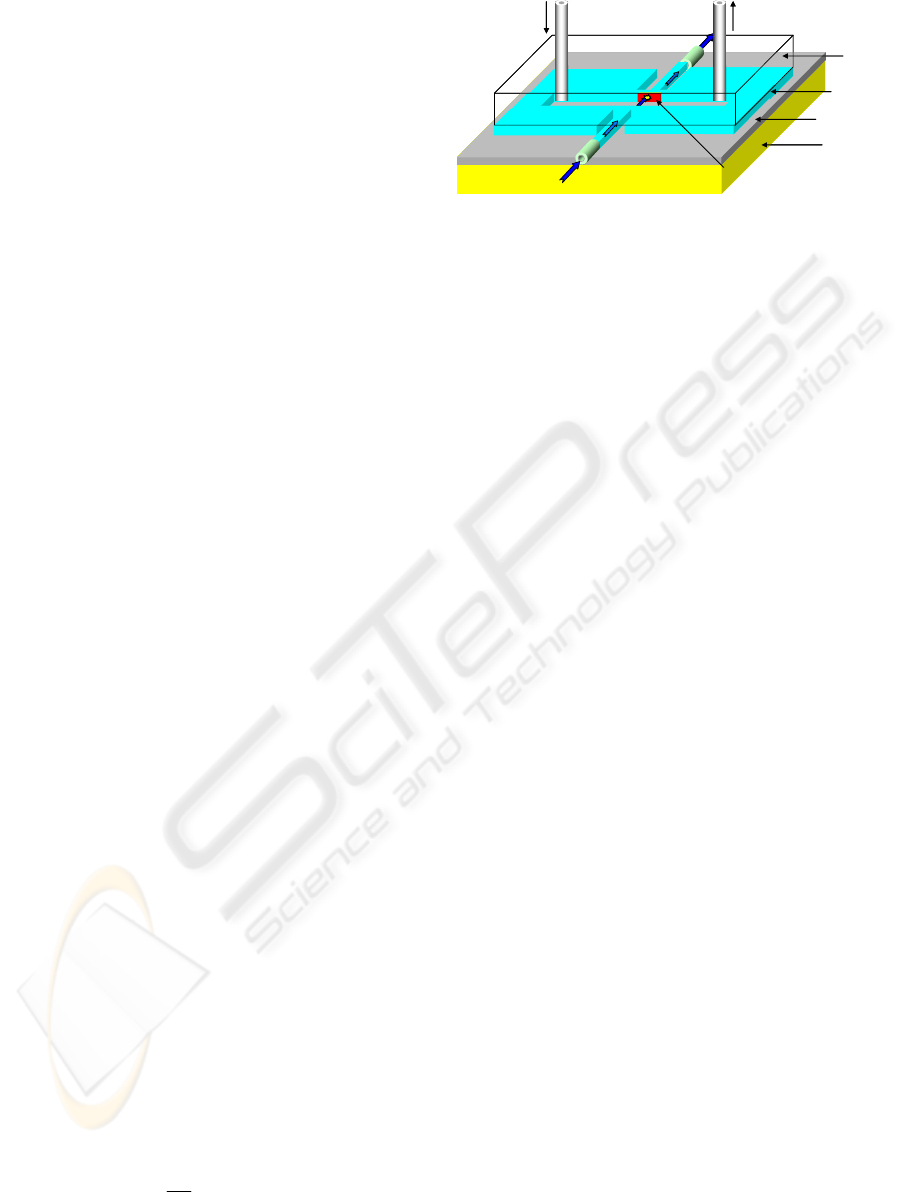
2 MATERIALS AND THEORY
2.1 Catechol
Catechol is a benzenediol, which is a subset of the
phenol class of organic compounds. The chemical
formula of Catechol is C
6
H
4
(OH)
2
.
Following
oxidation, catechol loses its hydrogen atoms from
the hydroxyl groups and becomes an
orthobenzoquinone, more commonly referred to as
an o-quinone. The oxidation of catechol in the cell
creates free radicals which cause damage to vital cell
components such as lipids, proteins and DNA (Sies,
1997).
2.2 Chitosan
Chitosan is a unique material that is well suited for
biological micro-devices due to its ability to be
selectivity deposited and its high density of amine
groups, which provide active bonding sites. The
selective deposition occurs due to chitosan’s
insolubility above a pH of 6.5. At low pH, chitosan
is protonated and soluble in water. As the pH rises
above 6.5, the amines lose their net positive charge
and the chitosan becomes insoluble. By taking
advantage of this property, one can deposit a film of
chitosan onto a cathode during an electrochemical
reaction. The pH rises with increasing proximity to
the cathode due to the reduction of the hydrogen
ions. The chitosan forms as a thin film or hydrogel
over the cathode surface depending on the amplitude
of the applied current density.
Chitosan has an added advantage over other
polysaccharides because it contains nucleophilic
primary amine (NH
2
) groups at nearly every
repeating sugar residue in its structure. The o-
quinones, which are formed from the oxidized
catechol molecules, bind to the amine groups and
impart physical changes to the film, such as a
change in the optical absorbance (Wu et al., 2005).
2.3 Optics
Understanding the operating principle of the device
requires a more detailed understanding of light
propagation and absorption through a medium. The
absorbance can be related to the concentration of
absorbing species present as demonstrated by the
Beer-Lambert Law:
lc
I
I
A
ε
=−= )(log
0
1
10
(1)
Fluid In
Fluid Out
Light In
Light Out
SU-8
Oxide
Deposited Chitosan Film
Silicon
PDMS
Fluid In
Fluid Out
Light In
Light Out
SU-8
Oxide
Deposited Chitosan Film
Silicon
PDMS
Figure 1: 3-d Schematic of the packaged device. The
device dimensions are 3.2 x 2.4 x 0.24 cm.
Where ε is the molar absorptivity, l is the path length
the light takes as it propagates through the absorbing
layer and c is the concentration. In our experiments,
the path length, l, is defined as the thickness of the
deposited chitosan film. O-quinones have been
reported to show a strong absorbance in the UV and
near UV range of the electromagnetic spectrum (Wu
et al., 2005). For this reason, a blue laser source at
472 nm was chosen for the optical measurements
taken with the MEMS sensor.
In our device, on-chip waveguides are patterned
from the polymer SU-8 as shown in the device
schematic (Figure 1). Blue light is coupled in and
out of the waveguides via multimode fibers with a
core diameter of 62.5 μm. The cross sectional area
of the polymer waveguides is 100 μm by 150 μm.
The light propagates through a film of chitosan that
has been deposited onto a transparent, conductive
film of indium tin oxide.
Since the absorbance measurement is purely
related to the optical power being received, it is
important to understand the different optical loss
mechanisms through the device in order to achieve
an acceptable signal to noise ratio. The primary
sources of loss are caused by waveguide losses
which include material absorption and scattering,
divergence of the light crossing the channel, and
roughness associated with the waveguide facets.
Waveguide loss will occur due to the roughness
of the waveguide and any material absorption
through the SU-8. This attenuation was measured to
be 21.15 dB/cm at 472 nm for waveguides with our
dimensions using image processing software. A top-
down digital photograph was taken of fabricated test
waveguides coupled to a blue laser source. The light
intensity down the length of the waveguide was
analyzed to determine the attenuation coefficient.
Divergence of the light as it crosses the
microfluidic channel from one waveguide to the next
is another possible source of loss. The light
capturing efficiency from one waveguide to the next
is found by integrating the surface energy of a beam
CATECHOL DETETION USING AN OPTICAL MEMS SENSOR
105