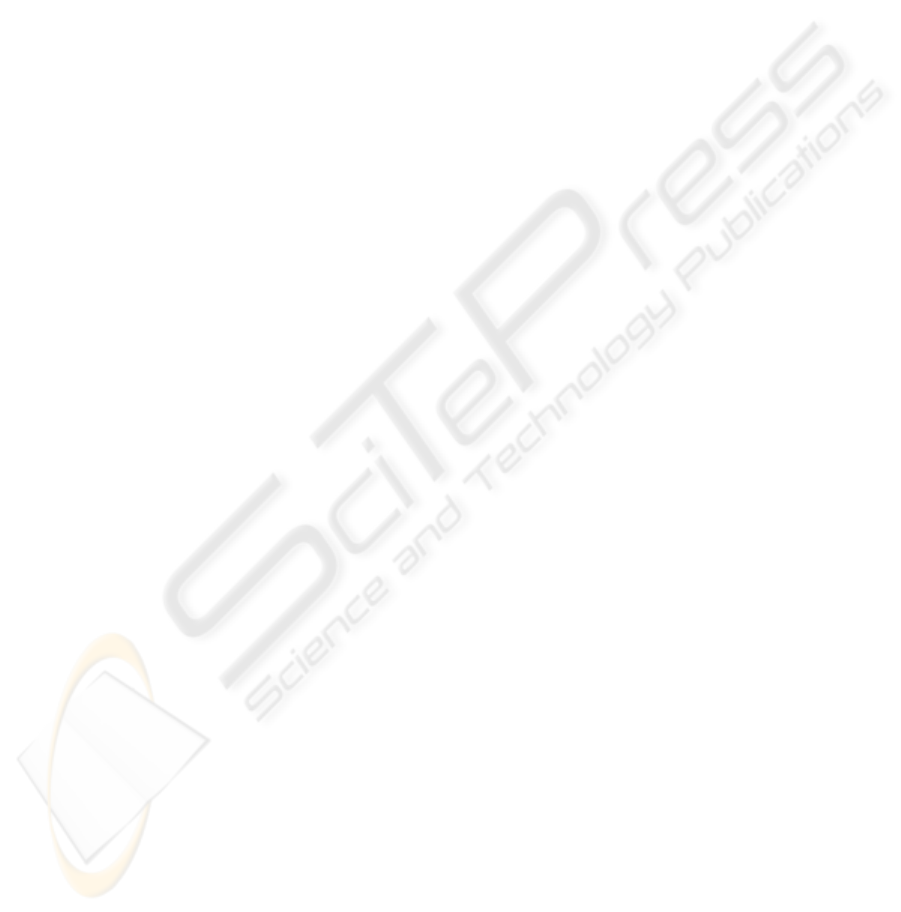
monitoring. Cardiac activity is clearly visible when a
person is lying prone on the floor.
Waveform correlation between all the test
subjects is clear when the recording posture is prone
(at both measurement heights) or supine (at
abdominal height). Respiration is most often visible
when recording is performed at abdominal height
while the person is lying prone or supine.
Combined with automatic fall detection, the vital
sign monitoring capability would be most useful in
many applications. These could include the care of
the elderly and seclusion monitoring. The limitations
in the favourable recording postures prevent the use
of this vital sign monitoring method in crucial
applications.
Our future work includes development of
algorithms for automatic detection of the best vital
sign recording point using the results obtained from
this study. We also aim to publish our existing
method for automatic fall detection.
ACKNOWLEDGEMENTS
This study was supported by the European Union and the
Jenny and Antti Wihuri Foundation. The authors are also
grateful to UPM Corporate Venturing for providing the
necessary multi-layer thick film sensor laminates.
REFERENCES
Rimminen, H., Linnavuo, M., and Sepponen, R., 2008.
Human Tracking Using Near Field Imaging. In
Proceedings of the Second International Conference
on Pervasive Health, pp. 148-151. ICST.
Prance, R. J., Debray, A., Clark, T. D., Prance, H., Nock,
M., Harland, C. J., and Clippingdale, A. J., 2000. An
ultra-low-noise electrical-potential probe for human-
body scanning. In Measurement Science and
Technology, Volume 11, Issue 3, pp. 291-297. Institute
of Physics Publishing.
Harland, C. J., Clark, T. D., and Prance, R. J., 2001.
Electric potential probes —New Directions in The
Remote Sensing of The Human Body. In
Measurement Science and Technology, Volume 13,
Issue 2, pp. 163-169. Institute of Physics Publishing.
Smith, W. J., and LaCourse, J. R., 2004. Non-Contact
Biopotential Recording from the Human Body Using a
Low-Impedance Charge Amplifier. In Proceedings of
the 30th Annual International Conference on
Bioengineering, pp. 31-32. IEEE.
Alametsä, J., Värri, A., Koivuluoma, M., and Barna, L.,
2004. The Potential of EMFi Sensors in Heart Activity
Monitoring. In 2nd OpenECG Workshop "Integration
of the ECG into the EHR & Interoperability of ECG
Device Systems”, pp. 1.-3.
Korjenevsky, A.V., 2004. Electric field tomography for
contactless imaging of resistivity in biomedical
applications. In Physiological Measurement, Volume
25, Issue 1. pp. 391-401. Institute of Physics
Publishing.
Tuykin, T.S., and Korjenevsky, A.V., 2007. Electric field
tomography system with planar electrode array. In
Proceedings of the 13th International Conference on
Electrical Bioimpedance and the 8th Conference on
Electrical Impedance Tomography, pp. 201-204.
Springer Berlin Heidelberg.
National Semiconductor Corporation, 1969. High Q Notch
Filter. In Linear Brief series, No. 5. National
Semiconductor.
Patterson, R.P., 1989. Fundamentals of impedance
cardiography. In Engineering in Medicine and Biology
Magazine, Volume 8, Issue 1, pp. 35-38. IEEE.
Vuorela, T., Vanhala, J., Seppä, V.-P., Hyttinen, J., 2008.
Two portable long-term measurement devices for ECG
and bioimpedance. In Proceedings of the Second
International Conference on Pervasive Health, pp.
169-172. ICST.
BIODEVICES 2009 - International Conference on Biomedical Electronics and Devices
130