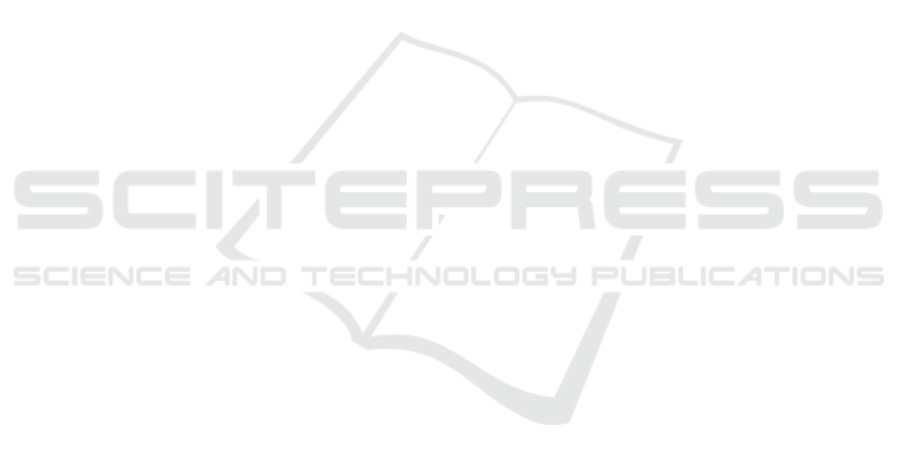
Brooke, J. (1996). Sus-a quick and dirty usability scale.
Usability evaluation in industry, 189(194):4–7.
Bujak, K. R., Radu, I., Catrambone, R., MacIntyre, B.,
Zheng, R., and Golubski, G. (2013). A psychological
perspective on augmented reality in the mathematics
classroom. Computers & Education, 68:536–544.
Cammeraat, S., Rop, G., and de Koning, B. B. (2020).
The influence of spatial distance and signaling on the
split-attention effect. Computers in Human Behavior,
105:106203.
de Jong, T. (2019). Moving towards engaged learning in
stem domains; there is no simple answer, but clearly a
road ahead. Journal of Computer Assisted Learning,
35(2):153–167.
de Jong, T., Ard Lazonder, Margus Pedaste, and Zacharias
Zacharia (2018). Simulations, games, and modeling
tools for learning. In Fischer, F., Hmelo-Silver, C. E.,
Goldman, S. R., and Reimann, P., editors, Interna-
tional Handbook of the Learning Sciences, pages 256–
266. Routledge, Taylor and Francis.
de Jong, T., Linn, M. C., and Zacharia, Z. C. (2013). Physi-
cal and virtual laboratories in science and engineering
education. Science (New York, N.Y.), 340(6130):305–
308.
Garz
´
on, J. and Acevedo, J. (2019). Meta-analysis of the im-
pact of augmented reality on students’ learning gains.
Educational Research Review, 27:244–260.
Ginns, P. (2006). Integrating information: A meta-analysis
of the spatial contiguity and temporal contiguity ef-
fects. Learning and Instruction, 16(6):511–525.
Husnaini, S. J. and Chen, S. (2019). Effects of guided in-
quiry virtual and physical laboratories on conceptual
understanding, inquiry performance, scientific inquiry
self-efficacy, and enjoyment. Physical Review Physics
Education Research, 15(1):31.
Ib
´
a
˜
nez, M.-B. and Delgado-Kloos, C. (2018). Augmented
reality for stem learning: A systematic review. Com-
puters & Education, 123:109–123.
Ivanjek, L., Schubatzky, T., Burde, J.-P., Hopf, M., Wil-
helm, T., Haagen-Sch
¨
utzenh
¨
ofer, C., Dopatka, L., and
Spatz, V. (2020). Development of a two-tier instrument
on simple electric circuits. Manuscript in preparation.
Janssen, J. and Kirschner, P. A. (2020). Applying collab-
orative cognitive load theory to computer-supported
collaborative learning: towards a research agenda.
Educational Technology Research and Development,
36(2):321.
Jones, K. A. and Sharma, R. S. (2019). An experiment in
blended learning: higher education without lectures.
International Journal of Digital Enterprise Technol-
ogy, 1(3):241.
Kapici, H. O., Akcay, H., and de Jong, T. (2019). Us-
ing hands-on and virtual laboratories alone or to-
gether—which works better for acquiring knowledge
and skills? Journal of Science Education and Technol-
ogy, 28(3):231–250.
Kapp, S., Thees, M., Strzys, M. P., Beil, F., Kuhn, J.,
Amiraslanov, O., Javaheri, H., Lukowicz, P., Lauer,
F., Rheinl
¨
ander, C., and Wehn, N. (2019). Augment-
ing kirchhoff’s laws: Using augmented reality and
smartglasses to enhance conceptual electrical experi-
ments for high school students. The Physics Teacher,
57(1):52–53.
Kuhn, J., Lukowicz, P., Hirth, M., Poxrucker, A., Wepp-
ner, J., and Younas, J. (2016). gphysics—using smart
glasses for head-centered, context-aware learning in
physics experiments. IEEE Transactions on Learning
Technologies, 9(4):304–317.
Leppink, J., Paas, F., van der Vleuten, C. P. M., van Gog,
T., and van Merri
¨
enboer, J. J. G. (2013). Development
of an instrument for measuring different types of cog-
nitive load. Behavior research methods, 45(4):1058–
1072.
Leppink, J., Paas, F., van Gog, T., van der Vleuten, C. P.,
and van Merri
¨
enboer, J. J. (2014). Effects of pairs of
problems and examples on task performance and dif-
ferent types of cognitive load. Learning and Instruc-
tion, 30:32–42.
Mayer, R. E., editor (2005). The Cambridge handbook of
multimedia learning. Cambridge Univ. Press, Cam-
bridge.
Mayer, R. E. (2009). Multimedia Learning. Cambridge
University Press, Cambridge.
Mayer, R. E. and Moreno, R. (2003). Nine ways to reduce
cognitive load in multimedia learning. Educational
Psychologist, 38(1):43–52.
Mayer, R. E., Moreno, R., Boire, M., and Vagge, S. (1999).
Maximizing constructivist learning from multimedia
communications by minimizing cognitive load. Jour-
nal of Educational Psychology, 91(4):638–643.
Mayer, R. E. and Pilegard, C. (2014). Principles for manag-
ing essential processing in multimedia learning: Seg-
menting, pre-training, and modality principles. In
Mayer, R. E., editor, The Cambridge handbook of
multimedia learning, Cambridge handbooks in psy-
chology, pages 316–344. Cambridge University Press,
New York.
Moreno, R. (2006). Learning in high-tech and multime-
dia environments. Current Directions in Psychological
Science, 15(2):63–67.
Pundak, D. and Rozner, S. (2008). Empowering engi-
neering college staff to adopt active learning meth-
ods. Journal of Science Education and Technology,
17(2):152–163.
Rau, M. A. (2020). Comparing multiple theories about
learning with physical and virtual representations:
Conflicting or complementary effects? Educational
Psychology Review, 16(3):183.
Santos, M. E. C., Chen, A., Taketomi, T., Yamamoto, G.,
Miyazaki, J., and Kato, H. (2014). Augmented reality
learning experiences: Survey of prototype design and
evaluation. IEEE Transactions on Learning Technolo-
gies, 7(1):38–56.
Schroeder, N. L. and Cenkci, A. T. (2018). Spatial conti-
guity and spatial split-attention effects in multimedia
learning environments: a meta-analysis. Educational
Psychology Review, 30(3):679–701.
Strzys, M. P., Kapp, S., Thees, M., Klein, P., Lukowicz,
P., Knierim, P., Schmidt, A., and Kuhn, J. (2018).
Physics holo.lab learning experience: using smart-
glasses for augmented reality labwork to foster the
The Effects of Augmented Reality: A Comparative Study in an Undergraduate Physics Laboratory Course
205