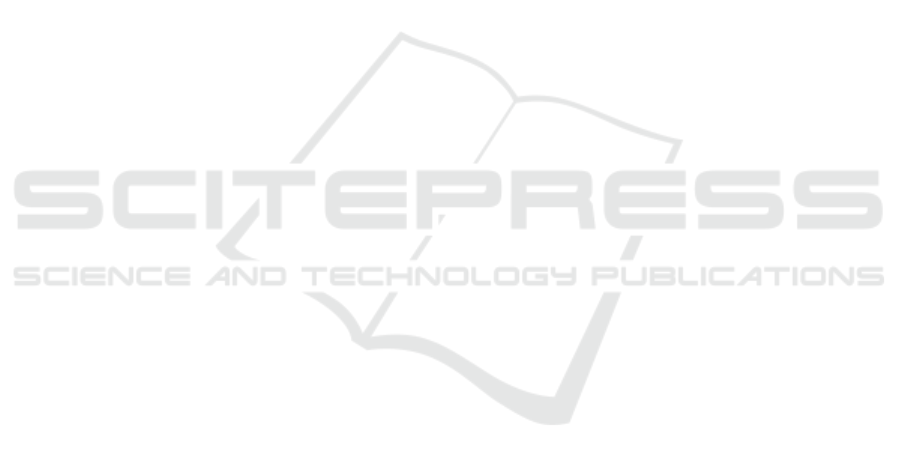
El Seoud, M. S. A. and Mady, A. S. (2019). A compre-
hensive review on volume rendering techniques. In
Proceedings of the 2019 8th International Conference
on Software and Information Engineering, ICSIE ’19,
page 126–131, New York, NY, USA. Association for
Computing Machinery.
Gardner, H. E. (2008). Multiple intelligences: New horizons
in theory and practice. Basic books.
Greil, G., Tandon, A. A., Silva Vieira, M., and Hussain, T.
(2017). Noninvasive imaging in adult congenital heart
disease. Frontiers in Pediatrics, 5(6):36.
Hegarty, M. (2014). Spatial thinking in undergraduate sci-
ence education. Spatial Cognition & Computation,
14(2):142–167.
Izard, S. G., Juanes, J. A., Pe
˜
nalvo, F. J. G., Estella, J. M. G.,
Ledesma, M. J. S., and Ruisoto, P. (2018). Virtual re-
ality as an educational and training tool for medicine.
Journal of medical systems, 42(3):50.
Konietschke, R., Tobergte, A., Preusche, C., Tripicchio, P.,
Ruffaldi, E., Webel, S., and Bockholt, U. (2010). A
multimodal training platform for minimally invasive
robotic surgery. In 19th International Symposium in
Robot and Human Interactive Communication, pages
422–427. IEEE.
Kumalasari, L., Yusuf Hilmi, A., and Priyandoko, D.
(2017). The application of multiple intelligence ap-
proach to the learning of human circulatory system.
In Journal of Physics Conference Series, volume 909.
Kurenov, S. N., Ionita, C., Sammons, D., and Demmy,
T. L. (2015). Three-dimensional printing to facilitate
anatomic study, device development, simulation, and
planning in thoracic surgery. The Journal of thoracic
and cardiovascular surgery, 149(4):973–979.
Maresky, H., Oikonomou, A., Ali, I., Ditkofsky, N.,
Pakkal, M., and Ballyk, B. (2018). Virtual real-
ity and cardiac anatomy: Exploring immersive three-
dimensional cardiac imaging, a pilot study in un-
dergraduate medical anatomy education. Clinical
Anatomy, 32.
Moore, R. A., Riggs, K. W., Kourtidou, S., Schneider,
K., Szugye, N., Troja, W., D’Souza, G., Rattan, M.,
Bryant III, R., Taylor, M. D., et al. (2018). Three-
dimensional printing and virtual surgery for congeni-
tal heart procedural planning. Birth defects research,
110(13):1082–1090.
Ong, C. S., Krishnan, A., Huang, C. Y., Spevak, P., Vricella,
L., Hibino, N., Garcia, J. R., and Gaur, L. (2018). Role
of virtual reality in congenital heart disease. Congen-
ital heart disease, 13(3):357–361.
Park, G., Lubinski, D., and Benbow, C. P. (2010). Recog-
nizing spatial intelligence. Scientific American.
Sajid, A., Ewy, G., Felner, J., Gessner, I., Gordon, M.,
Mayer, J., Shub, C., and Waugh, R. (1990). Cardiol-
ogy patient simulator and computer-assisted instruc-
tion technologies in bedside teaching. Medical educa-
tion, 24(6):512–517.
Silva, J. N., Southworth, M., Raptis, C., and Silva, J. (2018).
Emerging applications of virtual reality in cardiovas-
cular medicine. JACC: Basic to Translational Science,
3(3):420–430.
Standard, A. (2005). D2240,”standard test method for rub-
ber property—durometer hardness”. ASTM Interna-
tional, West Conshohoken, PA, USA.
Stout, K. K., Daniels, C. J., Aboulhosn, J. A., Bozkurt, B.,
Broberg, C. S., Colman, J. M., Crumb, S. R., Dearani,
J. A., Fuller, S., Gurvitz, M., Khairy, P., Landzberg,
M. J., Saidi, A., Valente, A. M., and Hare, G. F. V.
(2019). 2018 aha/acc guideline for the management
of adults with congenital heart disease: A report of the
american college of cardiology/american heart associ-
ation task force on clinical practice guidelines. Circu-
lation, 139(14):e698–e800.
Sun, Z., Lau, I., Wong, Y. H., and Yeong, C. H. Personalized
three-dimensional printed models in congenital heart
disease. Journal of Clinical Medicine, 8(522).
Triedman, J. K. and Newburger, J. W. (2016). Trends in
congenital heart disease. Circulation, 133(25):2716–
2733.
Vukicevic, M., Mosadegh, B., Min, J. K., and Little, S. H.
(2017). Cardiac 3d printing and its future directions.
JACC: Cardiovascular Imaging, 10(2):171–184.
3D Printing and 3D Virtual Models for Surgical and Percutaneous Planning of Congenital Heart Diseases
287