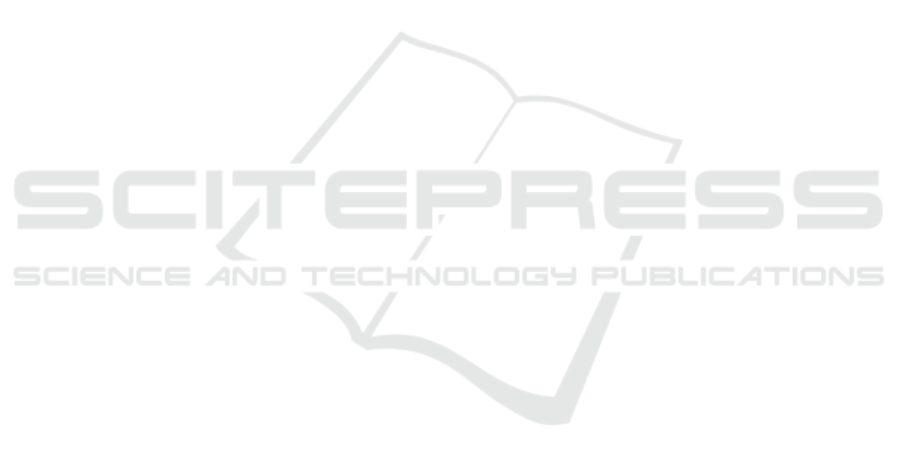
A REAL-TIME CELL PROLIFERATION AND MOTILITY
MONITORING SYSTEM
Nicola Moscelli, Sander van den Driesche, Michael J. Vellekoop
Institute of Sensors and Actuator Systems, Vienna University of Technology, Gusshausstrasse 27-29/E366, Vienna, Austria
Wojciech Witarski
Institute of Virology, Slovak Academy of Sciences, Dubravska Cesta 9, Bratislava, Slovak Republic
Keywords: Optical detection, CCD sensor, Real-time monitoring, Epithelial cells.
Abstract: In this contribution we present a compact imaging system to monitor the proliferation and the motility of
cells in real-time. Our monitoring system is compatible with standard multi-well plates and operates in CO
2
,
temperature and humidity controlled cell-culture incubators. Adherent grown epithelial cells in a multi-well
plate well, positioned on top of a custom made holder, have been monitored in real-time with a fixed CCD
image sensor. As light source an LED is placed above the plate holder. A field of view of 3.3 × 2.5 mm
2
was achieved by using a 4.6 × 4.0 mm
2
image sensor and mini lens system. The image sensor has a
resolution of 640 × 480 pixels. Consequently, the obtained sensing resolution of the imaging system is about
5 μm. The cell monitoring system has first been validated by visualizing micro-beads of known dimensions.
Then, our system has been successfully tested, tracking the migration paths and proliferation of respectively
adherent grown MDCK (Madin-Darby canine kidney) and A549 (human lung carcinoma) epithelial cells.
1 INTRODUCTION
The study of cell motility is of importance to
understand the mechanisms related to many
physiological and pathological processes such as
tumour progression and immunological responses
(Suresh, 2007). A commonly used technique to track
and quantify cell motility is by conducting gold
colloidal phagokinetic assays (Niinaka, 2001):
because of their movement, the adherently grown
cells displace gold monodisperse nanoparticles
deposited on the substrate, tracing clearly visible
paths. This technique is attractive for time-lapse
measurements, where the preservation of optimal
cultivation conditions such as fixed temperature,
humidity, oxygen and CO
2
levels for biological
culture growth is required. In order not to influence
the cultivation conditions during cell proliferation
and motility experiments, lab microscopes are
normally equipped with additional expensive stages
to control temperature, humidity, and CO
2
levels
(Poujade et al., 2007). Our proposed real-time
imaging system is a compact and low-cost
alternative to such an optical setup: it can be
operated in standard lab incubators and is also
compatible with unmodified lab disposables. Gabriel
et al. have presented a real-time cell monitoring
solution for incubator comprising a contact imaging
device. These devices are based on an image sensor
array directly in contact with the sample to be
investigated (Gabriel et al., 2009). However, this
proposed imaging device needs ad hoc modified lab
disposables and an elaborate cleaning step when
serial experiments are desired. Moreover, unlike
conventional optical imaging systems, where the
resolution depends on the pixel number and the lens
magnification, contact imaging devices have a
resolution which depends on the pixel size together
with the distance between the object and the sensor
surface (Ji et al., 2007). This limits the suitability of
such devices, because the sensor needs to be
decoupled by the biological sample to preserve its
functionality.
In the following section, we provide a
description of our monitoring system setup. In
Section 3 we present and discuss the validation tests
and the cell monitoring experiments.
230
Moscelli N., van den Driesche S., Vellekoop M. and Witarski W..
A REAL-TIME CELL PROLIFERATION AND MOTILITY MONITORING SYSTEM.
DOI: 10.5220/0003167002300233
In Proceedings of the International Conference on Biomedical Electronics and Devices (BIODEVICES-2011), pages 230-233
ISBN: 978-989-8425-37-9
Copyright
c
2011 SCITEPRESS (Science and Technology Publications, Lda.)