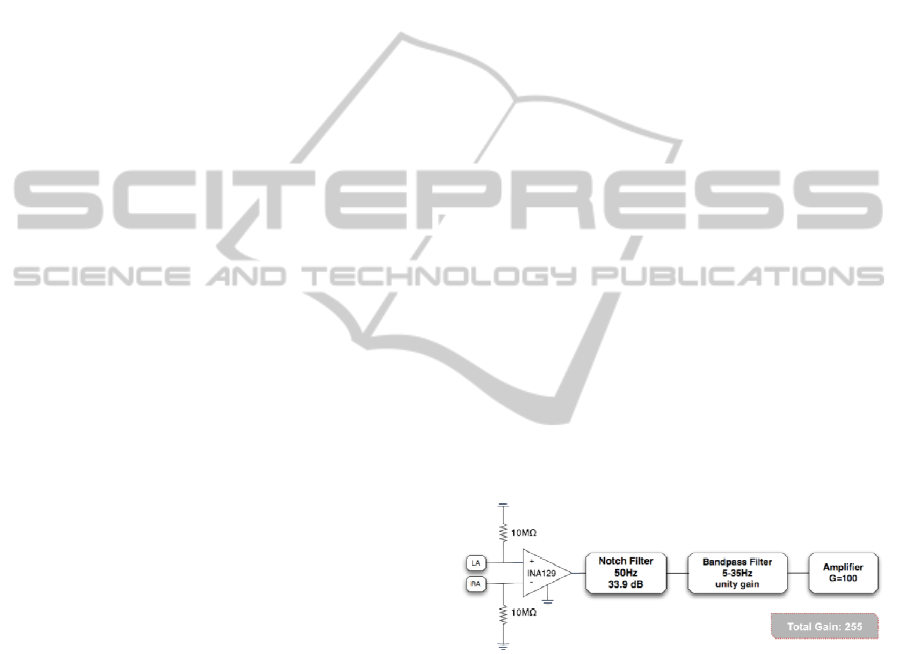
more difficult readouts. Three types of interface
between the electrode and the skin can be applied:
wet, dry/insulated and capacitive coupled. The first
one makes use of an electrolytic gel that helps to
promote the reduction of the contact impedance,
minimizing the risk of signal loss. This carries time-
consuming and complex procedures. The most
commonly used wet bioelectrode is the gel type
silver/silver chloride (Ag/AgCl), which can be found
both in reusable or disposable form. Dry and
insulated electrodes eliminate the need for an
electrolytic paste. The first type consist of a
biocompatible metal in direct contact with the skin,
being the coupling between them made by the user’s
sweat produced after it’s placement. On the other
hand, insulated electrodes are based on a dielectric
surface layer between the metal or semiconductor
and the skin. In this case, the bioelectric signal is
capacitively coupled between the skin and electrode,
without requiring electrical contact with the skin.
Some examples of dry/insulated electrodes and their
application can be found in (Baek et al., 2008; Ryu
et al., 2005). The third type of interface requires no
physical contact with the skin and it’s based on
capacitive pick-up electrodes. Basically, the
biopotential is obtained by capacitive coupling
between the body and the electrode, working both as
plates of a capacitor (Harland et al., 2002).
Bioelectric signals need to be amplified in order
to make them compatible with a variety of devices
such as A/D converters or display equipments. The
instrumentation amplifier is commonly used to
record biopotentials since it fulfils the basic
requirements for biopotential amplifiers, being
designed to have extremely large input impedance
and a small bias current. It works as a differential
amplifier, by applying high gain amplification
between signals at the positive and negative inputs.
Since the input signal of the amplifier consists of the
desired bioelectric signal and unwanted components
(e.g. power line interference signals, noise, etc.), it is
crucial to include a filtering stage. Generally, a
notch filter centered at 50 Hz (60 Hz in USA), and a
bandpass filter are used to remove these unwanted
signal components, that sometimes have higher
amplitudes than the desired bioelectric signal.
4 MEASUREMENTS
Measurements were carried in order to demonstrate
the concept of biopotential contactless recording.
The proof of concept consists into two stages: the
first experiment uses a conventional instrumentation
amplifier with subsequent filtering and amplification
stages; then, the resultant filtered and amplified
ECG is directly and contactless driven into a FET-
input instrumentation amplifier.
4.1 Experimental Setup
The modules used to validate our approach for
contactless detection of bioelectric signals include:
instrumentation amplifiers, notch filters, band-pass
filters and voltage amplifiers. The type of amplifiers
used, instrumentation amplifiers, need to fulfil a
particular set of requirements in order to provide
selective amplification to the biopotential, rejecting
the superimposed noise and interference
components:
- Have high input impedance (at least 10 MΩ)
and electrical isolation in order to inhibit
interference or distortion of the recorded signals.
- High CMRR (>80 dB according to (Neuman,
1998)) in order to separate as much as possible the
relevant signal from noise and interferences;
- Supply enough gain within its bandwidth in
order to reach an output level compatible with the
remaining system.
- Have low output impedance and supply the
amount of current necessary to the load.
- Provide protection to the patient from any
hazard of electrical shock.
Figure 2 shows the first module used in the
carried experiments.
Figure 2: First module comprising a conventional
acquisition circuit for biopotentials. LA corresponds to
Left Arm, and RA to right arm.
At this stage, an electrode was placed on each
arm, according to Lead I of Einthoven’s triangle,
resulting in a differential recording. The electrodes
were connected to both inputs of a precision
instrumentation amplifier (INA129, Texas
Instruments) with an input capacitance of 2 pF
(10
10
Ω) and a CMRR of 125 dB. The gain of the
amplifier was set to 155, by placing an RG of 320 Ω.
Since electrical circuits are usually interfered by ac
power lines, a notch filter was used to remove this
50 Hz interference, with an attenuation of 33.9 dB.
BIODEVICES 2011 - International Conference on Biomedical Electronics and Devices
132