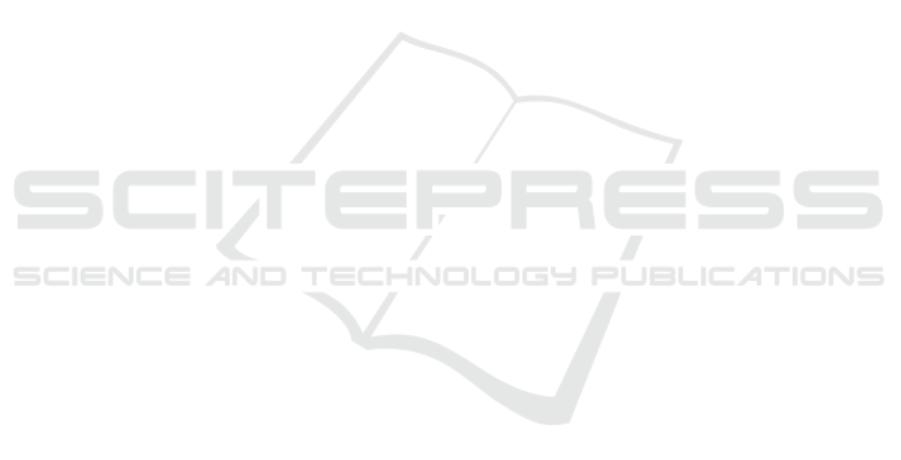
cal diseases were used to test the device. The acquired
images showed that it was possible to detect those
agents through images acquired via the µSmartScope,
which clearly illustrate the huge potential that this de-
vice can have, specially in developing countries with
limited access to healthcare services.
As future work, we want to tackle several of the
detected issues in order to achieve a more robust ver-
sion of the µSmartScope system. In particular, we
want to solve the significant negative impact of oc-
casional plastic imperfections (originated by the 3D
printing process) in the precision of the µStage, as
well as the currently low autonomy of the system for
continuous usage.
ACKNOWLEDGEMENTS
We would like to acknowledge the financial sup-
port from North Portugal Regional Operational Pro-
gramme (NORTE 2020), Portugal 2020 and the Euro-
pean Regional Development Fund (ERDF) from Eu-
ropean Union through the project ’Deus ex Machina:
Symbiotic Technology for Societal Efficiency Gains’,
NORTE-01-0145-FEDER-000026.
REFERENCES
Arpa, A., Wetzstein, G., Lanman, D., and Raskar, R. (2012).
Single lens off-chip cellphone microscopy. pages 23–
28. IEEE.
Cybulski, J. S., Clements, J., and Prakash, M. (2014). Fold-
scope: Origami-Based Paper Microscope. PLoS ONE,
9(6):e98781.
Dolgin, E. (2015). Portable pathology for Africa. IEEE
Spectrum, 52(1):37–39.
Krotkov, E. (1988). Focusing. International Journal of
Computer Vision, 1(3):223–237.
Liu, X., Wang, W., and Sun, Y. (2007). Dynamic evaluation
of autofocusing for automated microscopic analysis of
blood smear and pap smear. Journal of microscopy,
227(1):15–23.
Pirnstill, C. W. and Cot, G. L. (2015). Malaria Diagnosis
Using a Mobile Phone Polarized Microscope. Scien-
tific Reports, 5:13368.
Quinn, J., Andama, A., Munabi, I., and Kiwanuka, F.
(2014). Automated Blood Smear Analysis for Mobile
Malaria Diagnosis. In Mobile Point-of-Care Monitors
and Diagnostic Device Design, Devices, Circuits, and
Systems.
Rosado, L., Costa, J. M. C. d., Elias, D., and Cardoso, J. S.
(2016). A Review of Automatic Malaria Parasites
Detection and Segmentation in Microscopic Images.
http://www.eurekaselect.com, 14(1):11–22.
Shih, L. (2007). Autofocus survey: a comparison of algo-
rithms. In Electronic Imaging 2007, pages 65020B–
65020B. International Society for Optics and Photon-
ics.
Smith, Z. J., Chu, K., Espenson, A. R., Rahimzadeh, M.,
Gryshuk, A., Molinaro, M., Dwyre, D. M., Lane,
S., Matthews, D., and Wachsmann-Hogiu, S. (2011).
Cell-Phone-Based Platform for Biomedical Device
Development and Education Applications. PLoS
ONE, 6(3):e17150.
Sun, Y., Duthaler, S., and Nelson, B. J. (2005). Autofocus-
ing algorithm selection in computer microscopy. In
2005 IEEE/RSJ International Conference on Intelli-
gent Robots and Systems, pages 70–76. IEEE.
Switz, N. A., D’Ambrosio, M. V., and Fletcher, D. A.
(2014). Low-Cost Mobile Phone Microscopy with a
Reversed Mobile Phone Camera Lens. PLoS ONE,
9(5):e95330.
Tenenbaum, J. M. (1970). Accommodation in computer vi-
sion. phd dissertation. Technical report, DTIC Docu-
ment.
Tseng, D., Mudanyali, O., Oztoprak, C., Isikman, S. O.,
Sencan, I., Yaglidere, O., and Ozcan, A. (2010). Lens-
free microscopy on a cellphone. Lab on a Chip,
10(14):1787–1792.
Utzinger, J., Becker, S. L., Knopp, S., Blum, J., Neu-
mayr, A. L., Keiser, J., and Hatz, C. F. (2012). Ne-
glected tropical diseases: diagnosis, clinical manage-
ment, treatment and control. Swiss Medical Weekly,
142:w13727.
Wakerly, M. (2012). Usb serial for android.
WHO, W. H. O. (1991). Basic malaria microscopy.
Zachary, G. (2015). Technology alone won’t improve health
in Africa [Spectral lines]. IEEE Spectrum, 52(1):7–7.
BIODEVICES 2017 - 10th International Conference on Biomedical Electronics and Devices
48